Chemical thermodynamics explains how energy transforms within reactions and systems. It rests on fundamental laws that describe energy conservation, the increase of entropy, and system stability at equilibrium. You’ll learn about key properties like internal energy, enthalpy, and free energy, which help predict whether reactions happen spontaneously. Understanding these principles can clarify material behavior, energy flow, and practical applications in industry and environment. Keep exploring to uncover how thermodynamics shapes the world around you.
Key Takeaways
- Chemical thermodynamics studies energy transformations during chemical reactions and phase changes.
- It introduces key concepts like enthalpy, entropy, and free energy to predict reaction spontaneity.
- The laws of thermodynamics explain energy conservation and the direction of spontaneous processes.
- State functions such as internal energy and Gibbs free energy depend only on current system conditions.
- Thermodynamics applications include designing industrial processes, energy systems, and understanding material behavior.
Fundamental Laws Governing Thermodynamics
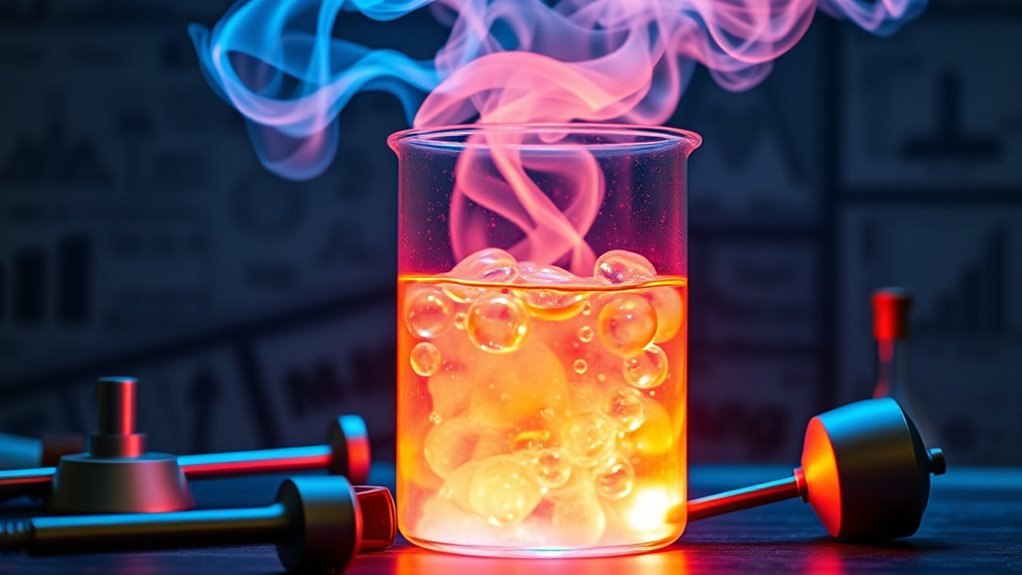
The fundamental laws of thermodynamics describe how energy transforms and how systems evolve over time. You’ll learn that energy can’t be created or destroyed; it only changes form, which is essential to understanding energy interactions. AI security considerations are increasingly relevant when analyzing complex systems and their safety protocols. The first law, also known as conservation of energy, tells you that the total energy in a closed system remains constant, with energy flowing as heat or work. This law helps you determine the maximum useful work obtainable from a system. The second law states that entropy, or disorder, tends to increase, meaning spontaneous processes favor higher entropy. The third law indicates that as temperature approaches absolute zero, entropy approaches zero, providing an absolute scale for entropy. Energy interactions are fundamental to the study of thermodynamics and help explain how different systems exchange energy. Together, these laws form the foundation for analyzing energy and system behavior across sciences.
Core State Functions and Their Significance
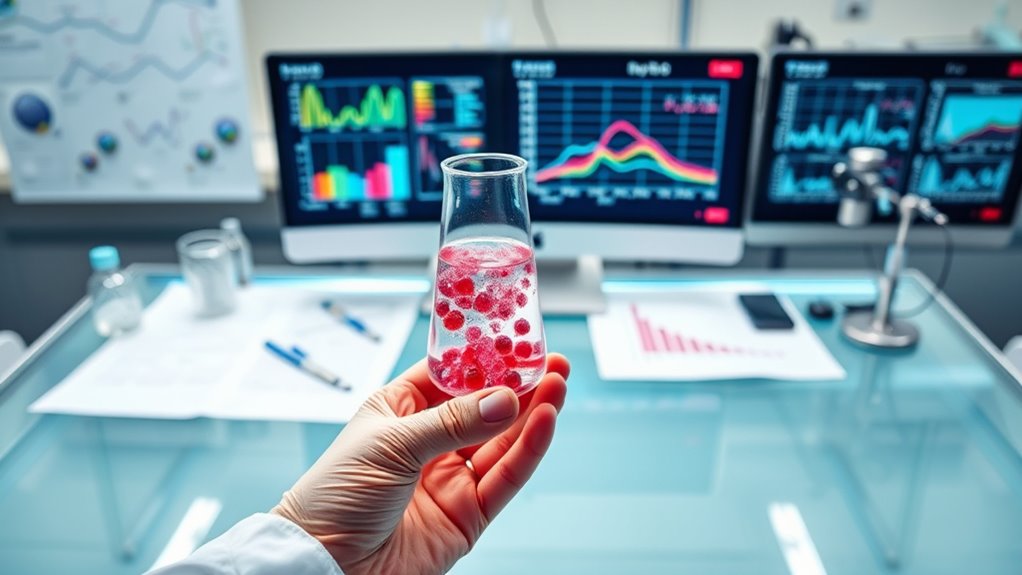
Understanding core state functions is essential because they describe properties of a system that depend only on its current condition, not on how it arrived there. Internal energy (U) reflects the total energy within the system, including molecular motions and positions, and changes in U (∆U) indicate energy transfer via heat and work. Enthalpy (H) accounts for internal energy plus pressure-volume work, helping analyze heat flow at constant pressure. Entropy (S) measures disorder and reflects the number of microstates, increasing in spontaneous processes. Free energies like Gibbs (G) and Helmholtz (A) determine spontaneity and maximum work output, depending only on the system’s state. Understanding these properties allows scientists to predict system responses how systems will respond to changes and to design processes that are energetically favorable. Additionally, recognizing the significance of proper thermodynamic measurements is crucial for accurate modeling and process optimization in various scientific and engineering applications. Recognizing the role of state functions also helps in simplifying complex thermodynamic calculations and analyses, especially when analyzing fundamental properties that depend solely on the system’s current state.
Understanding Spontaneous Processes and Equilibrium
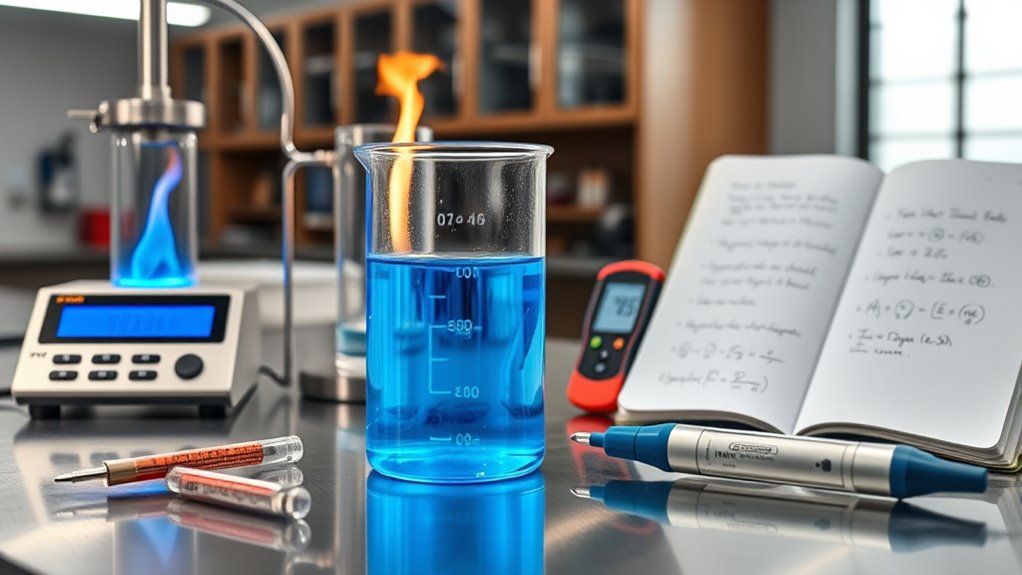
Core state functions like entropy and free energy help explain why certain processes happen spontaneously. When a process increases entropy (ΔS > 0), it tends to be spontaneous, but there are exceptions. The key indicator is the Gibbs free energy change (ΔG); if it’s negative, the process is spontaneous. Additionally, understanding for-sale 100 can provide context for practical applications of thermodynamics in various industries. Spontaneous processes release energy, often as heat or work, and lead to more stable, irreversible states. They occur naturally without external input and push the system toward equilibrium, where ΔG equals zero, indicating no net change. Conditions such as temperature and pressure influence spontaneity, helping you predict whether a process will proceed on its own or require external energy. Recognizing thermodynamic spontaneity is crucial for designing efficient energy systems and chemical reactions. Furthermore, advancements in automation technology have improved our ability to model and predict these spontaneous changes more accurately. Additionally, processes involving phase transitions are often driven by spontaneous changes in free energy, which are essential in many industrial applications. Understanding the relationship between entropy and energy is fundamental to grasping how spontaneous processes occur.
Energy Changes in Chemical Reactions
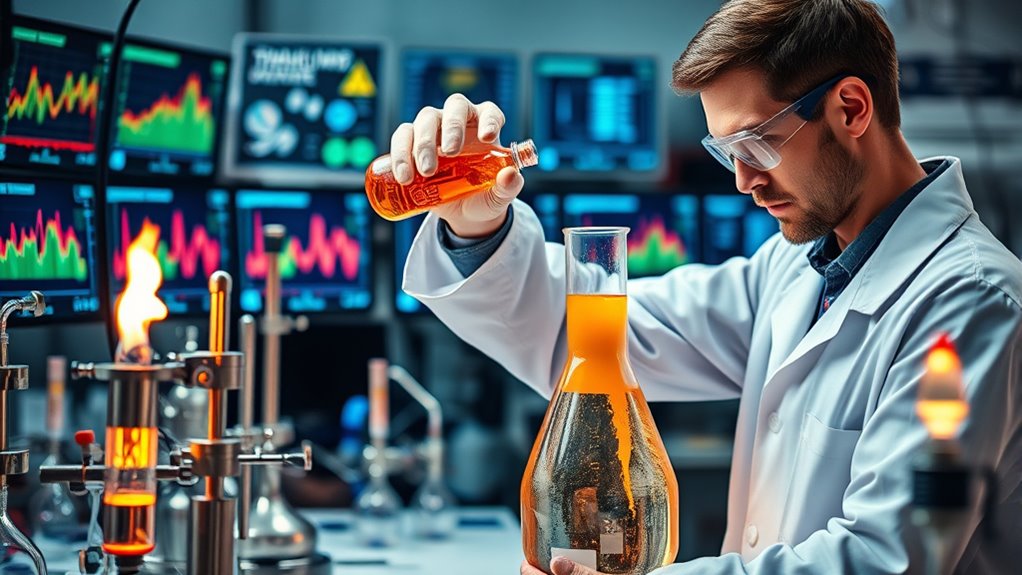
Energy changes in chemical reactions involve the transfer of heat between the system and its surroundings, which can be measured and analyzed to understand reaction behavior. Reactions are classified as exothermic if they release net energy, resulting in a temperature increase, or endothermic if they absorb net energy, causing cooling. Bond breaking requires energy input, while bond formation releases energy; the balance determines whether a reaction is exothermic or endothermic. Bond energies help quantify these processes. Bond energy is measured in units of kJ/mol, providing a quantitative measure of bond strength. Calorimetry and Hess’s Law assist in measuring and calculating these energy changes accurately. Understanding the energy transfer during reactions is essential for predicting reaction outcomes and designing efficient chemical processes. Additionally, understanding the relationship between reaction spontaneity and energy changes can aid in optimizing chemical reactions for industrial applications.
Practical Applications of Thermodynamics in Chemistry
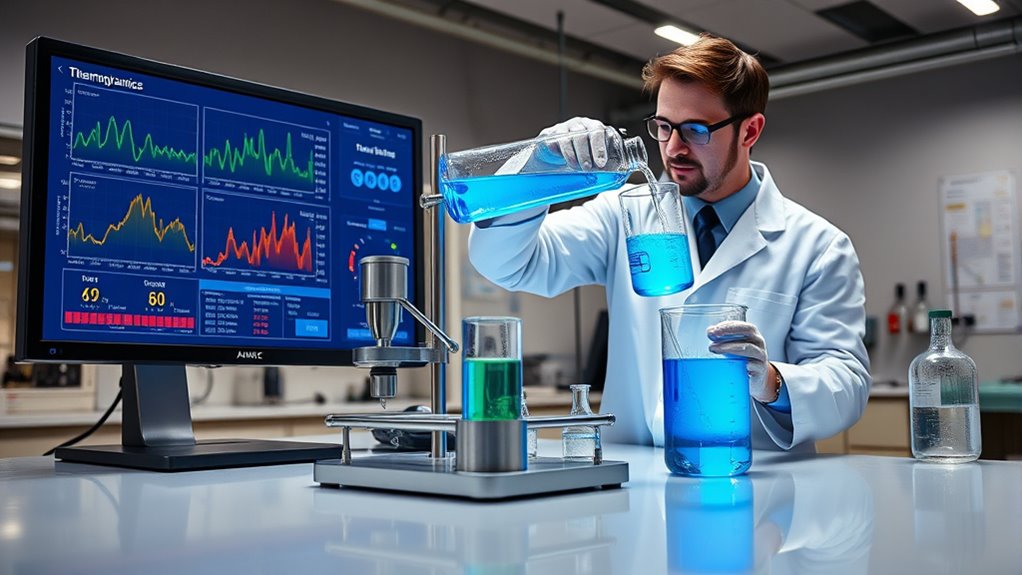
Thermodynamics plays a vital role in guiding practical applications across various fields of chemistry, enabling scientists and engineers to optimize processes and improve efficiency.
In industry, you use thermodynamics to enhance reactions like the Haber process, adjusting pressure and temperature to maximize ammonia yield. Refrigeration cycles rely on thermodynamic principles to evaluate energy efficiency through the coefficient of performance.
Biofuel production involves enthalpy and entropy analysis to optimize yields during transesterification. Waste heat recovery systems convert excess heat into electricity, boosting energy efficiency. Thermodynamic principles are also essential in developing sustainable energy solutions and improving existing technologies.
In environmental solutions, Gibbs free energy analysis guarantees the stability of carbon capture and storage. Thermodynamics also informs the design of fuel cells, electrolysis, geothermal energy, and material synthesis, helping you develop sustainable, cost-effective technologies.
Additionally, understanding the high nutritional value of chia seeds can aid in developing healthier dietary options and functional foods. Incorporating glycolic acid products into skincare routines exemplifies how thermodynamic principles can optimize cosmetic formulations for better skin absorption and effectiveness. Moreover, considering the biochemical pathways involved in these processes can further enhance the efficiency and sustainability of chemical applications.
Common Misconceptions and Limitations of Thermodynamic Principles
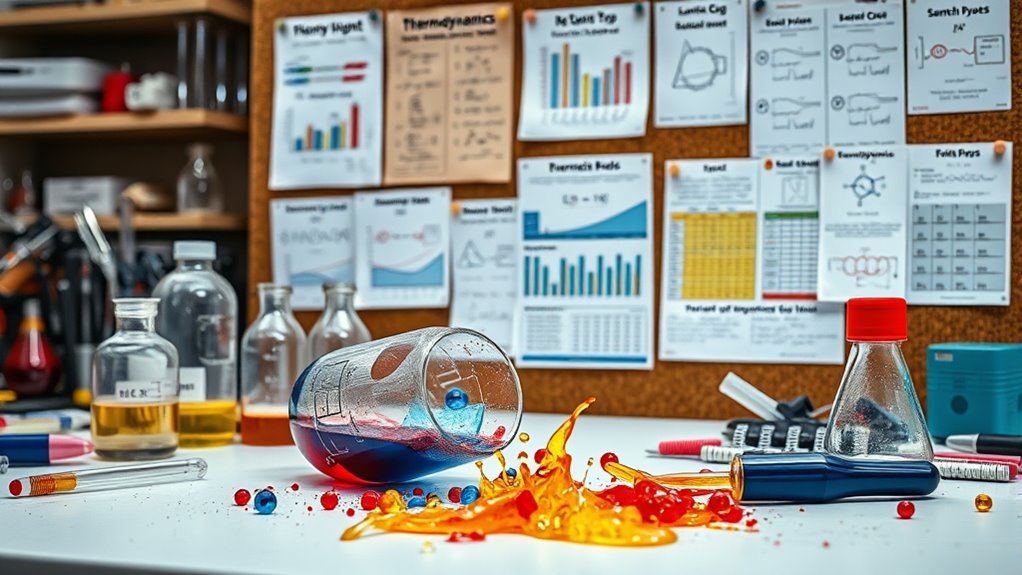
Many common misconceptions about thermodynamic principles can lead to misunderstandings of how systems actually behave. You might think temperature directly equals average molecular kinetic energy, but molecules store potential energy in bonds, affecting internal energy. This explains why kinetic theory often mismatches specific heat measurements.
Temperature doesn’t directly equal average molecular kinetic energy; potential energy in bonds also impacts internal energy.
Similarly, you may believe Brownian motion results solely from random collisions, but bond interactions and potential energy fluctuations also influence particle movement.
Another misconception is that phase transitions happen because vibrations increase; in reality, energy redistributes into potential energy, weakening bonds and causing latent heat absorption.
Additionally, the First Law focuses only on energy conservation, ignoring energy quality and irreversibility.
Furthermore, kinetic theory falters under extreme conditions, needing corrections like Van der Waals equations to account for molecular volume and forces.
Moreover, understanding the role of intermolecular forces is crucial for accurately describing phase behavior and deviations from ideality.
The Role of Thermodynamics in Material and Environmental Science
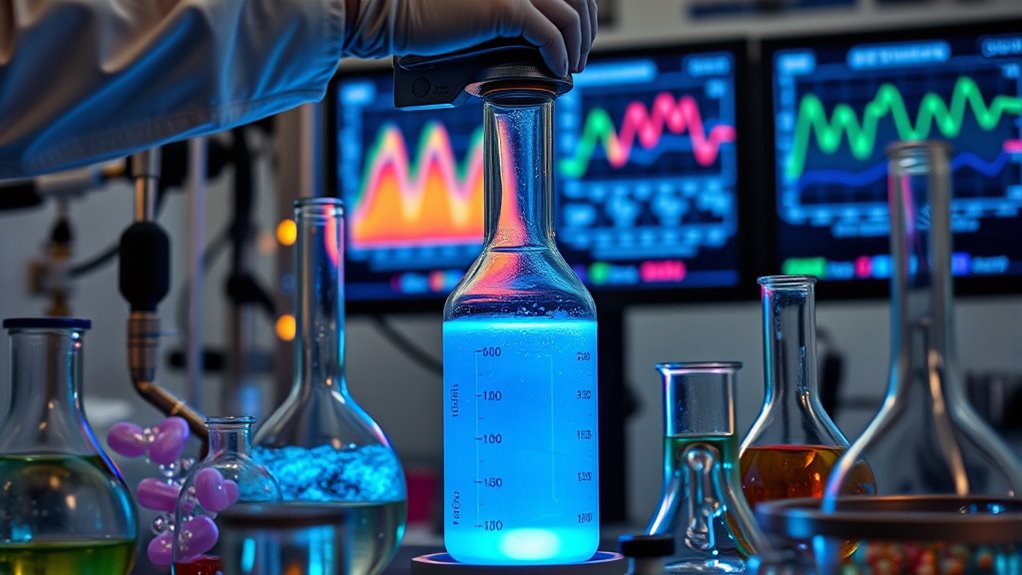
Understanding how systems behave under different conditions is essential for advancing materials and environmental science. Thermodynamics helps you analyze material properties, such as stability and reactivity, by applying concepts like entropy and enthalpy. Entropy indicates the level of disorder within a system, influencing how materials respond to changes in temperature and pressure. When selecting materials, consider their thermal characteristics to optimize durability and performance. Knowledge of phase transitions enables you to predict how materials change state, improving manufacturing processes. Thermodynamic cycles allow you to evaluate energy efficiency, ensuring designs serve their purpose effectively. In environmental science, thermodynamics guides sustainable practices, from recycling processes to pollution reduction. It also supports climate modeling and ecosystem analysis by understanding energy flows. Additionally, thermodynamics drives innovation in developing advanced materials and energy storage systems, contributing to technological progress and environmental stewardship.
Frequently Asked Questions
How Do Temperature and Pressure Influence Spontaneity in Real Systems?
Think of temperature and pressure as the tide shaping the course of a boat. You can steer reactions toward spontaneity by raising temperature, which amplifies entropy’s role.
Or by adjusting pressure to favor volume changes. In real systems, both factors work together, shifting equilibrium and influencing reaction direction.
You need to take into account their combined effects to understand whether a process will naturally proceed or require energy input.
Can Entropy Decrease Locally Without Violating the Second Law?
You wonder if entropy can decrease locally without breaking the second law. It can, as long as the overall entropy of the universe increases.
When you use external energy, like in refrigerators or biological systems, you reduce entropy in one area, but this is balanced by a greater increase elsewhere.
What Is the Role of Entropy in Biological Systems?
You might think entropy always increases, but in biological systems, it plays a crucial role by enabling life processes. You see, enzymes reduce entropic barriers, speeding up reactions.
Cells manage entropy by maintaining order internally while dissipating excess to the environment. This balance allows you to understand how organisms sustain complexity and adapt, using free energy to control disorder, ensuring survival despite the universal trend toward increasing entropy.
How Do Kinetic Factors Affect Reaction Spontaneity Predictions?
You might think kinetic factors influence reaction spontaneity, but they don’t. Kinetics determines how fast a reaction occurs, while thermodynamics predicts if it’s spontaneous based on Gibbs free energy.
Even if a reaction is slow due to high activation energy, it can still be spontaneous if it’s thermodynamically favored. Catalysts can speed up reactions without changing their spontaneity, so kinetics affects rate but not whether the reaction will happen spontaneously.
Are Thermodynamic Principles Applicable to Open, Non-Isolated Systems?
Yes, thermodynamic principles definitely apply to open, non-isolated systems. You need to take into account how energy and matter exchange affect the system’s state variables like pressure, temperature, and composition.
These exchanges influence whether processes occur spontaneously or require energy input. By analyzing energy conservation (First Law) and entropy changes (Second Law), you can predict system behavior and understand how open systems reach equilibrium or steady states.
Conclusion
You’ve just revealed the secrets of chemical thermodynamics—powerful enough to control the universe’s tiniest particles! Mastering these principles lets you predict reactions, harness energy, and even influence the environment with unmatched precision. It’s not just science; it’s the key to transforming the world around you! So dive deep, challenge misconceptions, and unleash your potential—because understanding thermodynamics could be the most revolutionary knowledge you’ll ever possess!